The Problem With Modern Exercise Science
On average physiological responses to exercise are relatively predictable. For example, resistance training increases strength and muscle hypertrophy, energy system training improves endurance, and so forth. However, modern exercise science still lacks the precision to determine the optimal volume, intensity, duration, frequency, and timing of exercise stimuli to optimally enhance a specific individual's health, fitness, and performance.
“It is wise to note that we are all individuals and that whereas physiological responses to particular stimuli are largely predictable, the precise responses and adaptations to those stimuli will vary among individuals. Therefore, the same training regimen may not equally benefit all those who follow it.” -George Brooks
Truthfully, applied exercise science has not advanced much closer to the aforementioned ideals since the early 2000s when George Brooks wrote the above quote. To move towards a greater understanding of personalized, dose-response optimized exercise, we need rigorous systematic research that…
elucidates the optimal dose (i.e., volume and intensity), duration, frequency, and timing of exercise stimuli to elicit specific adaptations;
Intentionally co-administers potentially agonistic and antagonistic stimuli;
Assesses the influence of modifiers of training-induced adaptation such as age, training status, genetics, comorbidities, and drug use.
However, underpowered studies, poor controls, and lack of funding make it difficult to conduct research that addresses the above concerns. Beyond that, there are more significant issues at play, including the fact that many exercise science studies only measure end-responses from training (i.e., whole system adaptations such as increases in strength, muscle hypertrophy, or task-specific endurance). This last point is crucial, but we'll need to lay some foundations before unpacking that further.
Understanding Exercise Induced Adaptations
Physiological adaptations are changes that occur within individuals in response to external factors like exercise or environmental factors such as altitude. In the history of adaptation research, one of the earliest proposed ideas was the concept of overload by Julius Wolff, who linked the loading of bones to their adaptation in the late 1800s. His hypothesis was later extended to other organs, and the term overload was expanded to include forms of loading that weren't mechanical. However, while Wolff's general principle was correct in that exercise is indeed required for exercise-induced adaptations, it didn't explain the underlying mechanisms by which said adaptations occurred. It wasn't until a different theory, called the super-compensation theory, was proposed that anyone provided a potential mechanistic explanation for adaptation.
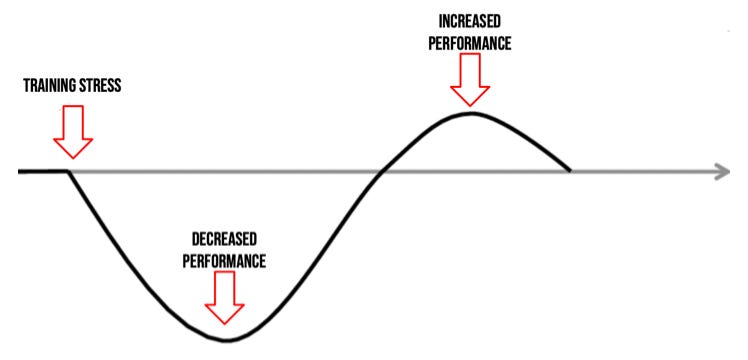
The super-compensation theory is based on Hans Seyle's general adaptation syndrome (GAS) concept. Seyle's GAS concept is characterized by a decline in an often undefined y-axis variable during exercise followed by its recovery following exercise. According to the super-compensation theory, recovery of the y-axis variable does not just reach pre-exercise levels but overshoots it, as demonstrated in the image above.
The super-compensation theory is widely accepted among exercise scientists, strength and conditioning coaches, and athletes. However, despite being so widely cited, it has many flaws and lacks clear mechanistic justification. Moreover, in recent years the little scientific explanation that does exist has largely crumbled under the weight of newer evidence. In fact, Hans Seyle's GAS syndrome concept had already lost favor among stress physiologists even before the super-compensation theory came to prominence. John Kiely summed up the major critiques with Selye’s theory in a paper titled Periodization Theory: Confronting an Inconvenient Truth where he states, “As the twentieth century entered its final quarter, the explanatory limitations of Hans Selye’s paradigm were increasingly exposed. Most notably, the portrayal of stress as a predictable biologically mediated phenomenon was undermined by the demonstrable effects of non-physical factors on physiological stress responses and increasingly convincing evidence that stress responses were not generalized and non-specific, but highly individualized and context-specific.”
Furthermore, contemporary research invalidates the super-compensation theories assertions which state that recovery periods are essential for physiological adaptation to occur. For example, the heart adapts to exercise despite continuous contraction, and skeletal muscles can adapt and grow in response to chronic electrical stimulation applied continuously over weeks. Despite what many popular training-related books say, there is little to no evidence that the super-compensation time course is necessary for exercise adaptation. Additionally, in contract, hundreds of scientific references support an alternative hypothesis stating that signal transduction pathways mediate all adaptations to exercise.
According to signal transduction theory, exercise affects the body by activating different genetic, epigenetic, and metabolomic expression circuits that shape physical, cognitive, and behavioral traits. The gist of this process is as follows: exercise leads to the up-regulation, or release, of ligands (ions, signaling molecules, etc.) that bind specific target receptors, resulting in downstream signaling events. These downstream signaling events vary based on the particular receptor that is activated. For example, when a ligand binds a G-protein coupled receptor, the receptor changes conformation, causing its subunits to separate. The α-subunit activates one downstream target, whereas the ß,y-subunits activate another downstream target. The resulting effects of these signaling cascades include gene transcription, gene translation, protein synthesis, and protein breakdown. Ultimately these processes results in tissues, organs, organ systems, or organisms that have adapted to exercise.
Now that we’ve covered the basics of exercise adaptation, we can expand our aperture and encapsulate a broader range of related topics.
Dose-Response Relationships
In pharmacology, we have concepts called efficacy and potency. Efficacy refers to the effectiveness of a drug independent of its concentration. For example, drug B is considered more efficacious than drug A if it can elicit a greater maximal response. Potency, on the other hand, is a relative term and refers to the effectiveness of a drug at a given concentration. For example, drug A is more potent than drug B if it elicits a greater response at a specific dose. The concepts of efficacy and potency are depicted in the image below:
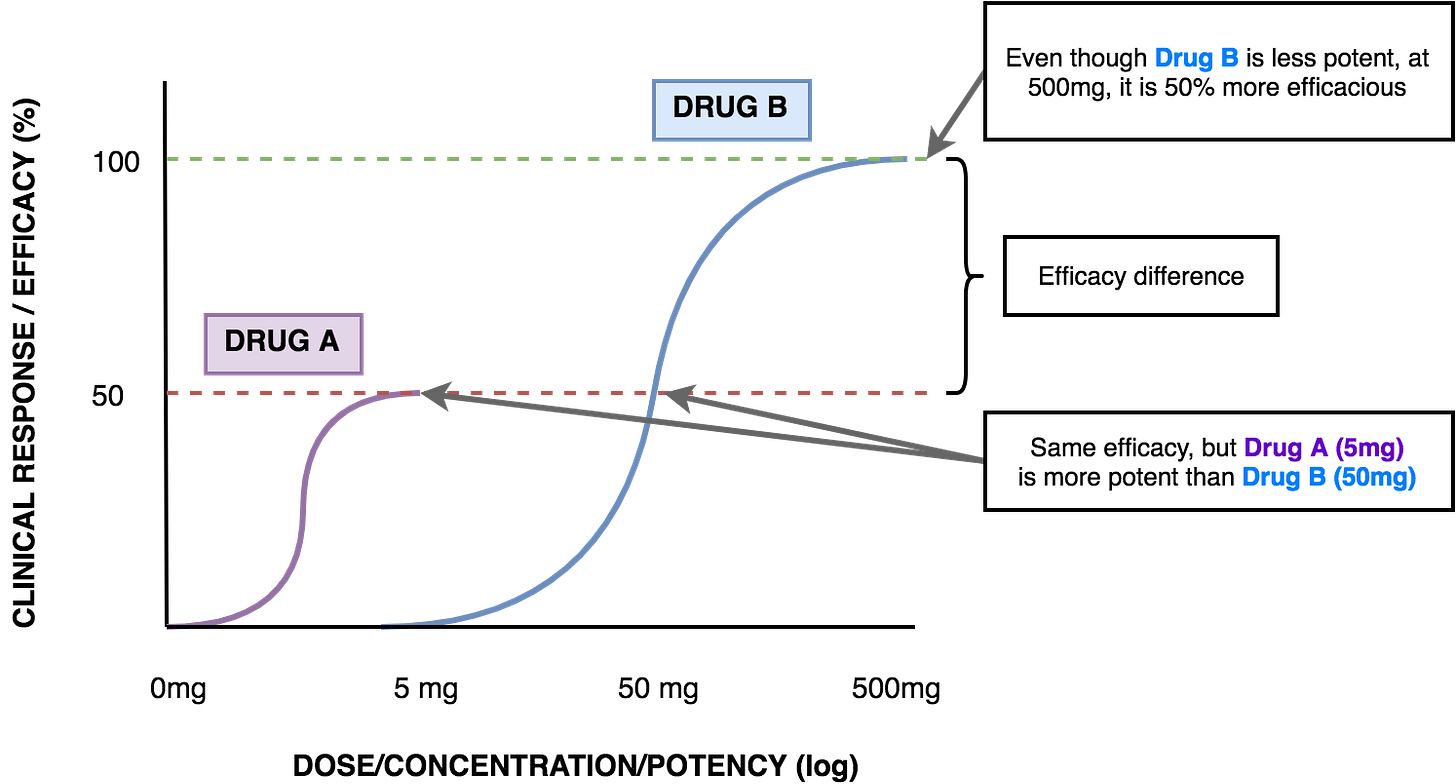
It’s intuitive to think that a drug which is more efficacious is also more potent and vice versa. However, this is not always the case. In the image above you can see that drug A is more potent that drug B, despite being less efficacious. For example, drug A elicits a 50% maximal response at 5mg whereas drug B elicits a 50% maximal response at 50mg. However, drug A never elicits more than a 50% maximal response, whereas drug B is capable of eliciting an 100% response at high doses. Thus, drug B is more potent.
The concepts of efficacy and potency may seem disconnected from exercise science. However, they also apply to exercise-induced stimuli. After all, exercise exerts an effect on the body by up-regulating the release of endogenous ligands which bind receptors. Drugs are exogenous ligands, but ultimately exert their effects on the body through the same process.
Amplification Masks The Efficacy And Potency Of Exercise Stimuli
Now, lets compare two theoretical dose-response curves for hack squats and leg presses. Which one is more efficacious and more potent on a per-set basis?
On the low and high end of the volume spectrum, it’s challenging to determine which exercise is more efficacious and/or potent. However, at intermediate volumes (2-4 sets), the leg press is both more efficacious and potent for this trainee. It’s important to note that these comparisons on sets 2-4 are relative. Both exercises are equally efficacious, in an absolute sense, since they can exert the same maximal response. However, the leg press is the more potent since a maximal response is achieved with slightly less training volume.
This all sounds perfect in theory, but reality is never this simple. For starters, we don’t have an easy way to measure an individual’s exercise response. Additionally, in exercise science, we don’t even have a standardized definition of what an exercise-induced adaptive response entails. For example, do we define an exercise-induced response as the change in receptor expression, the activation of second messenger systems, or the big-picture functional response, such as the change in muscle hypertrophy that occurs over time? Presumably, the latter is what you care about the most. Unfortunately, this is the most difficult adaptive response to get a clean measurement of since the time course of measurable effect can be days, weeks, or months depending on the trainee’s fitness level (whereas you can measure RNA micro-expression before and after a workout and calculate the direct change resulting from exercise).
In a perfect world, you can control many factors to determine the precise effects of a given training method on functional outcomes such as strength, endurance, or muscle hypertrophy. However, this still does little to help us understand the efficacy and potency of a given method. The reason for this is that the further in time your measurements occur from the stimulus, the more amplification of signals there is.
On the left-hand side of the image above, you can see two stimuli of varying weights (efficacies). As you scan the picture from left to right, you can see a fulcrum and three pairs of dose-response curves representing the two stimuli at varying time points. The first time point represents released signaling molecules binding their receptors, the second time point represents the activation of second messenger systems, and the third time point represents the functional adaptation to the stimulus. You'll also find two horizontal dotted lines. The lower dotted line represents the threshold of measurement effect for the stimuli, and the upper dotted line represents the maximal response threshold.
Now, let's say the larger stimulus in yellow represents a leg press while the smaller stimulus in red represents a hack squat. The closer we observe to the fulcrum, the easier it is to see how these two stimuli vary in their efficacies. On the contrary, the further we observe from the fulcrum, the harder it is to observe any meaningful difference. For example, these two different exercise stimuli may cause very different acute physiological responses. Still, we may not observe a difference in functional training adaptations over a multi-week controlled trial. Thus, if we only measured the functional training response, we would have no real way of knowing which of the two exercises is more efficacious.
Standardizing Measurements Of Exercise Adaptations
If we want exercise science to move forward, we must standardize how we define, measure, and compare training responses. I suggest that we classify training responses into three groups. Group 1 measures the cellular responses to exercise, group 2 measures the acute physiological responses to exercise, and group 3 measures the functional changes that occur in response to exercise. For any given set of exercise interventions, you can compare the responses across these three types of standardized tests, as demonstrated below:
An example of a group 1 measurement would be assessing RNA microexpression levels before and after an exercise stimulus to determine which method impacts acute gene expression to the greatest degree for a given individual. For example, the image below is from a 12-week study where participants performed a low-volume resistance training protocol 3x/week (36 total workouts). Baseline muscle biopsy measurements were taken before the 12 weeks, and the follow-up muscle biopsy was taken after the 12 weeks. Below you can see the VEGFa expression levels pre and post-intervention for each individual:
Note that the untrained individuals in this study saw a significant increase in VEGFa expression after the 12-week intervention, whereas the trained individuals did not. In fact, some of the trained individuals saw a decrease in VEGFa expression levels. Imagine performing a similar analysis comparing pre/post-intervention RNA expression levels for a given individual to determine which training method is most effective for eliciting a specific adaptation. By doing so, you could make informed inferences about the efficacy and potency of a given stimulus. However, it doesn’t always tell you which method leads to the most significant end effect, so we still want to measure functional responses as well.
Whereas group 1 measurements quantity cellular responses to exercise, group 2 measurements assess acute physiological responses such as changes in blood lactate concentrations, muscle oxygenation, active nitric oxide, and other metabolism biomarkers. These measurements are helpful for understanding how different training interventions impact an individual’s physiology. Additionally, you can easily correlate group 2 measurements with group 1 and group 3 measurements to create a reliable real-time indicator for personalized, dose-response optimized exercise.
Finally, group 3 measurements assess the functional response to exercise, such as increased strength, endurance, muscle hypertrophy, or power. Traditional performance assessments all fall under this category, and in effect, group 3 measurements are intended to answer the question of how a training intervention impacts performance. However, group 3 measurements do not tell you about the efficacy or potency of said methods, so they should be performed in conjunction with group 1 and 2 measurements.
Pharmacology As A Confounding Factor
Drugs (legal or otherwise) can act as agonists, antagonists, or modulators of physiological responses. An agonist compound can increase the efficacy or potency of a stimulus, and an antagonist can decrease the efficacy or potency of a stimulus. Again, this is important to consider because certain pharmacological compounds can wash out the differences between two training interventions, making it impossible to determine which is more efficacious and/or potent. This is most pronounced in enhanced athletes. For example, anabolic androgenic steroids can bind nuclear receptors and exert their own downstream effects, thus lowering the required threshold for an exercise stimulus to exert a maximal response. This is why you can have geared athletes using suboptimal training methodologies but still making exceptional progress (this phenomenon is most easily observed in untested bodybuilding).
It’s also important to consider how antagonists can impact exercise adaptation. For example, the H2 histamine receptor is an important mediator of exercise-induced adaptations. Anti-histamines are strong antagonists of said receptors and may act as negative modulators of adaptation. Thus, any drugs (including medications or supplements) have the potential to alter the efficacy and/or potency of a given training stimulus and should be accounted for as best as possible when investigating dose-response relationships of various training methodologies.
A New Direction For Human Performance
What i’m proposing in this article is a radical departure from how exercise science is currently practiced. However, I don’t think it’s so far left field as to not be achievable. In fact, DARPA has been hard at working trying to accomplish the goals I outlined at the start of this article for years:
“The crux of MBA is correlating the externally observable physical, behavioral, and cognitive features and traits of highly successful specialized operators — their phenotypes — with measurable elements of their biology to understand and ultimately anticipate how they might perform in various situations over time; this information can then be used to improve training and development at an individual level.” -Source
In essence, this entails understanding how exercise impacts an individual at the micro (cellular) and macro (whole system) scale. Once that is achieved we can identify biomarkers that correlate with acute cellular changes and/or whole-system adaptations to exercise. Ideally, these biomarkers are integrated in non-invasive wearable devices, which will allow for real-time biosensor feedback to guide exercise based on known personalized dose-response curves.
Interested In Learning More?
If you’re interested in learning more you can check out free Ebook, Paradigm Shift: Rethinking Human Performance.
Great article!
I have been thinking about the idea of finding a minimal dose-response relationship for some time now and found the concept of 'critical slowing down' from complex systems theory, which would be super interesting when applied to measuring individual response.
https://iopscience.iop.org/article/10.1209/0295-5075/132/18001
The fact that every system transitioning from one state to another exhibit an increase in variability is a fascinating concept to consider, and I am just wondering whether it might be applicable within the context of human performance. I guess that finding data that provides the necessary resolution to detect these nuanced changes will be hard; however, there have been some attempts using detrended fluctuation analysis (DFA) to find long-term memory in sports data. I have personally played with some biomechanical data such as stride-to-stride variability and vertical oscillations, but I wonder if time-series variability could be a viable direction for establishing individual adaptation thresholds. It is clear that it is impossible to find global predictors; however, at the level of individual limiters, it might be interesting. I am curious to hear your thoughts on this.
Feel free to reach out, and thanks again for sharing your valuable insights.
https://www.linkedin.com/in/mat%C4%9Bj-n-a74788b3/
Thank you again !